Abstract
Electron dynamics in water are of fundamental importance for a broad range of phenomena1,2,3, but their real-time study faces numerous conceptual and methodological challenges4,5,6. Here we introduce attosecond size-resolved cluster spectroscopy and build up a molecular-level understanding of the attosecond electron dynamics in water. We measure the effect that the addition of single water molecules has on the photoionization time delays7,8,9of water clusters. We find a continuous increase of the delay for clusters containing up to four to five molecules and little change towards larger clusters. We show that these delays are proportional to the spatial extension of the created electron hole, which first increases with cluster size and then partially localizes through the onset of structural disorder that is characteristic of large clusters and bulk liquid water. These results indicate a previously unknown sensitivity of photoionization delays to electron-hole delocalization and indicate a direct link between electronic structure and attosecond photoionization dynamics. Our results offer new perspectives for studying electron-hole delocalization and its attosecond dynamics.
This is a preview of subscription content,access via your institution
Relevant articles
Open Access articles citing this article.
Apparatus for attosecond transient-absorption spectroscopy in the water-window soft-X-ray region
Scientific ReportsOpen Access21 February 2023
Atomic partial wave meter by attosecond coincidence metrology
Nature CommunicationsOpen Access29 August 2022
Access options
访问其他自然组合journa性质和54ls
Get Nature+, our best-value online-access subscription
$29.99 per month
cancel any time
Subscribe to this journal
Receive 51 print issues and online access
$199.00 per year
only $3.90 per issue
Rent or buy this article
Get just this article for as long as you need it
$39.95
Prices may be subject to local taxes which are calculated during checkout
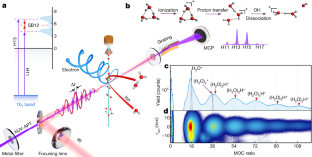

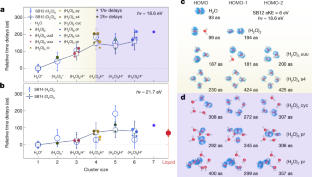
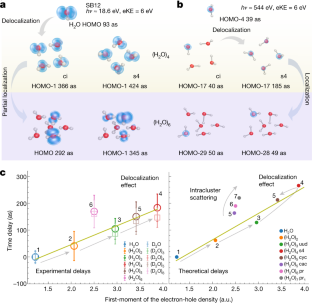
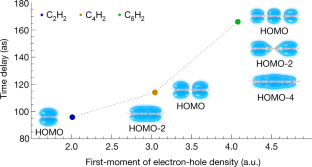
Data availability
Source dataare provided with this paper.
Code availability
All non-standard code used to analyse the data is available from the corresponding author upon reasonable request.
References
Sanche, L. Beyond radical thinking.Nature461, 358–359 (2009).
Boudaiffa, B., Cloutier, P., Hunting, D., Huels, M. A. & Sanche, L. Resonant formation of DNA strand breaks by low-energy (3 to 20 eV) electrons.Science287, 1658–1660 (2000).
Garrett, B. C. et al. Role of water in electron-initiated processes and radical chemistry: issues and scientific advances.Chem. Rev.105, 355–390 (2005).
Svoboda, V. et al. Real-time observation of water radiolysis and hydrated electron formation induced by extreme-ultraviolet pulses.Sci. Adv.6, eaaz0385 (2020).
Loh, Z.-H. et al. Observation of the fastest chemical processes in the radiolysis of water.Science367, 179–182 (2020).
Jordan, I. et al. Attosecond spectroscopy of liquid water.Science369, 974–979 (2020).
Schultze, M. et al. Delay in photoemission.Science328, 1658–1662 (2010).
Klünder, K. et al. Probing single-photon ionization on the attosecond time scale.理论物理。Rev. Lett.106, 143002 (2011).
Huppert, M., Jordan, I., Baykusheva, D., von Conta, A. & Wörner, H. J. Attosecond delays in molecular photoionization.理论物理。Rev. Lett.117, 093001 (2016).
Alizadeh, E., Orlando, T. M. & Sanche, L. Biomolecular damage induced by ionizing radiation: the direct and indirect effects of low-energy electrons on DNA.Annu. Rev. Phys. Chem.66, 379–398 (2015).
Marsalek, O. et al. Chasing charge localization and chemical reactivity following photoionization in liquid water.J. Chem. Phys.135, 224510 (2011).
安德森,p . w .缺乏在某些ra扩散ndom lattices.理论物理。Rev.109, 1492–1505 (1958).
Hunt, P., Sprik, M. & Vuilleumier, R. Thermal versus electronic broadening in the density of states of liquid water.Chem. Phys. Lett.376, 68–74 (2003).
Prendergast, D., Grossman, J. C. & Galli, G. The electronic structure of liquid water within density-functional theory.J. Chem. Phys.123, 014501 (2005).
Pieniazek, P. A., Sundstrom, E. J., Bradforth, S. E. & Krylov, A. I. Degree of initial hole localization/delocalization in ionized water clusters.J. Phys. Chem. A.113, 4423–4429 (2009).
Winter, B. et al. Full valence band photoemission from liquid water using EUV synchrotron radiation.J. Phys. Chem. A.108, 2625–2632 (2004).
Pohl, M. N. et al. Do water’s electrons care about electrolytes?Chem. Sci.10, 848–865 (2019).
Dörner, R. et al. Cold target recoil ion momentum spectroscopy: a ‘momentum microscope’ to view atomic collision dynamics.理论物理。Rep.330, 95–192 (2000).
Ullrich, J. et al. Recoil-ion and electron momentum spectroscopy: reaction microscopes.Rep. Prog. Phys.66, 1463–1545 (2003).
Shiromaru, H., Shinohara, H., Washida, N., Yoo, H. & Kimura, K. Synchrotron radiation measurements of appearance potentials for (H2O)2+, (H2O)3+, (H2O)2H+and (H2O)3H+in supersonic jets.Chem. Phys. Lett.141, 7–11 (1987).
Dong, F., Heinbuch, S., Rocca, J. J. & Bernstein, E. R. Dynamics and fragmentation of van der Waals clusters: (H2O)n, (CH3OH)n, and (NH3)nupon ionization by a 26.5 eV soft X-ray laser.J. Chem. Phys.124, 224319 (2006).
Baykusheva, D. & Wörner, H. J. Theory of attosecond delays in molecular photoionization.J. Chem. Phys.146, 124306 (2017).
Dahlström, J. M., L’Huillier, A. & Maquet, A. Introduction to attosecond delays in photoionization.J. Phys. B: At. Mol. Opt. Phys.45, 183001 (2012).
Barth, S. et al. Valence ionization of water clusters: from isolated molecules to bulk.J. Phys. Chem. A113, 13519–13527 (2009).
Jordan, I. & Wörner, H. J. Extracting attosecond delays from spectrally overlapping interferograms.J. Opt.20, 024013 (2018).
Shi, Z., Ford, J. V., Wei, S. & Castleman, A. W. Water clusters: contributions of binding energy and entropy to stability.J. Chem. Phys.99, 8009–8015 (1993).
Liu, K., Cruzan, J. D. & Saykally, R. J. Water clusters.Science271, 929–933 (1996).
Temelso, B., Archer, K. A. & Shields, G. C. Benchmark structures and binding energies of small water clusters with anharmonicity corrections.J. Phys. Chem. A.115, 12034–12046 (2011).
Malloum, A., Fifen, J. J., Dhaouadi, Z., Nana Engo, S. G. & Conradie, J. Structures, relative stability and binding energies of neutral water clusters, H2On,n = 2-30.New J. Chem.43, 13020–13037 (2019).
Gianturco, F. A., Lucchese, R. R. & Sanna, N. Calculation of low-energy elastic cross sections for electron-CF4scattering.J. Chem. Phys.100, 6464–6471 (1994).
Natalense, A. P. P. & Lucchese, R. R. Cross section and asymmetry parameter calculation for sulfur 1sphotoionization of SF6.J. Chem. Phys.111, 5344–5348 (1999).
Rescigno, T. N., Lengsfield III, B. H. & McCurdy, C. W. inModern Electronic Structure TheoryVol. 1 (ed. Yarkony, D. R.) 501–588 (World Scientific, 1995).
Rescigno, T. N., McCurdy, C. W., Orel, A. E. & Lengsfield III, B. H. inComputational Methods for Electron-Molecule Collisions(eds Huo, W. & Gianturco, F.) 1–44 (Springer, 1995).
Orel, A. E. & Rescigno, T. N. Variational expressions for first-order properties involving continuum wave functions.理论物理。启一个41, 1695–1697 (1990).
Silvestri, F. & Marrocchi, A. Acetylene-based materials in organic photovoltaics.Int. J. Mol. Sci.11, 1471–1508 (2010).
Jahnke, T. et al. Ultrafast energy transfer between water molecules.Nat. Phys.6, 139–142 (2010).
Mucke, M. et al. A hitherto unrecognized source of low-energy electrons in water.Nat. Phys.6, 143–146 (2010).
Zhang, P., Perry, C., Luu, T. T., Matselyukh, D. & Wörner, H. J. Intermolecular Coulombic decay in liquid water.理论物理。Rev. Lett.128, 133001 (2022).
Unger, I. et al. Observation of electron-transfer-mediated decay in aqueous solution.Nat. Chem.9, 708–714 (2017).
Hermann, G. et al. Orbkit: a modular python toolbox for cross-platform postprocessing of quantum chemical wavefunction data.J. Comput. Chem.37, 1511–1520 (2016).
Acknowledgements
We thank A. Schneider and M. Seiler for their technical support. We gratefully acknowledge funding from an European Research Council Consolidator grant (project no. 772797-ATTOLIQ), project no. 200021_172946 as well as the NCCR-MUST, funding instruments of the Swiss National Science Foundation. D.J. thanks the European Union’s Horizon 2020 programme (FP-RESOMUS – MSCA 801459) program for a fellowship and A. Schild for introduction to ORBKIT. X.G. thanks the National Natural Science Foundation of China (grant nos. 12122404 and 11974114) for financial support. Most of the theoretical results have been obtained on the ETH Zürich Euler cluster and the NCCR-Cluster supercomputer. The basis-set complex Kohn variational calculations, obtained at the Lawrence Berkeley National Laboratory (LBNL), were realized with the support of the US Department of Energy (DOE) under contract no. DE-AC02-05CH11231. Calculations performed there made use of the resources of the National Energy Research Scientific Computing Centre, a DOE Office of Science User Facility, and the Lawrencium computational cluster resource provided by the IT Division at the LBNL.
Author information
Authors and Affiliations
Contributions
X.G. and S.H. carried out the experiments and analysed the experimental data. X.G. constructed the experimental apparatus with contributions from S.H., K.Z. and C.P. D.J. performed most of the theoretical calculations. R.L. realized the basis-set complex Kohn variational calculations. X.G., S.H. and H.J.W wrote the initial manuscript. All authors discussed and reviewed the manuscript.
Corresponding author
Ethics declarations
Competing interests
The authors declare no competing interests.
Peer review
Peer review information
Naturethanks the anonymous reviewers for their contribution to the peer review of this work.Peer reviewer reportsare available.
Additional information
Publisher’s note施普林格自然仍neutral with regard to jurisdictional claims in published maps and institutional affiliations.
Extended data figures and tables
Extended Data Fig. 1 Effect of the channel coupling on photoionization delays of the 1b1band.
a, Calculated time delays for photoionization out of the 1b1band of water clusters relative to H2O for a kinetic energy of eKE = 6.0 eV (SB12). The results of the single-channel calculations using ePolyScat30,31(filled circles) are compared with multi-channel calculations using the basis-set complex Kohn method32,33,34(filled triangles) are compared. The experimental results are identical to those shown in Fig.3.b, Same asa, but calculated/measured for SB14.
Extended Data Fig. 2 Effect of orbital delocalization on photoionization delays of the 3a1band.
Correlation between the photoionization delays and the first moment of the electron-hole density of the 3a1-orbital-band of water monomer and tetramer (s4) for a kinetic energy of eKE = 6 eV.
扩展数据图3分子的几何效应on orbital delocalization and photoionization delays of (H2O)4s4.
The structures are obtained from AIMD time propagation at different times (see legend). AIMD are run using density-functional theory, the Turbomole software package, the B3-LYP density functional, the def2-TZVP basis and a temperature ofT = 100 K.
Supplementary information
Supplementary Information
Supplementary text, figures and references.
Rights and permissions
Springer Nature or its licensor holds exclusive rights to this article under a publishing agreement with the author(s) or other rightsholder(s); author self-archiving of the accepted manuscript version of this article is solely governed by the terms of such publishing agreement and applicable law.
About this article
Cite this article
Gong, X., Heck, S., Jelovina, D.et al.Attosecond spectroscopy of size-resolved water clusters.Nature609, 507–511 (2022). https://doi.org/10.1038/s41586-022-05039-8
Received:
Accepted:
Published:
Issue Date:
DOI:https://doi.org/10.1038/s41586-022-05039-8
This article is cited by
Apparatus for attosecond transient-absorption spectroscopy in the water-window soft-X-ray region
Scientific Reports(2023)
Atomic partial wave meter by attosecond coincidence metrology
Nature Communications(2022)
Comments
By submitting a comment you agree to abide by ourTermsandCommunity Guidelines. If you find something abusive or that does not comply with our terms or guidelines please flag it as inappropriate.